Emotional and cognitive changes in chronic kidney disease
Article information
Abstract
Chronic kidney disease (CKD) leads to cognitive impairment and emotional changes. However, the precise mechanism underlying the crosstalk between the kidneys and the nervous system is not fully understood. Inflammation and cerebrovascular disease can influence the development of depression in CKD. CKD is one of the strongest risk factors for cognitive impairment. Moreover, cognitive impairment occurs in CKD as patients experience the dysregulation of several brain functional domains due to damage caused to multiple cortical regions and to subcortical modulatory neurons. The differences in structural brain changes between CKD and non-CKD dementia may be attributable to the different mechanisms that occur in CKD. The kidney and brain have similar anatomical vascular systems, which may be susceptible to traditional risk factors. Vascular factors are assumed to be involved in the development of cognitive impairment in patients with CKD. Vascular injury induces white matter lesions, silent infarction, and microbleeds. Uremic toxins may also be directly related to cognitive impairment in CKD. Many uremic toxins, such as indoxyl sulfate, are likely to have an impact on the central nervous system. Further studies are required to identify therapeutic targets to prevent changes in the brain in patients with CKD.
INTRODUCTION
Chronic kidney disease (CKD) is a critical health burden worldwide. The overall prevalence rate of CKD in Korea is 8.2% [1]. A number of risk factors, including diabetes, hypertension, and hyperlipidemia, are responsible for the progression of CKD and cardiovascular events [2,3]. Moreover, CKD itself is one of the strongest risk factors for cardiovascular events. These risk factors lead to endothelial cell injury, which eventually results in end organ damage [4]. Recent studies have examined the mechanism of the crosstalk between the kidney and other organs [5,6]. Kidney injury can adversely affect the function and induce pathological changes in the gut, lungs, heart, brain, and the immune system [7–9].
Under normal physiological conditions, homeostasis is maintained by the communication between the kidney and the central nervous system (CNS). For example, under normal physiological conditions, systemic changes in osmolarity are detected by osmoreceptors in the CNS. When osmolarity is high, vasopressin is secreted from the hypothalamus and the posterior region of the pituitary gland. Vasopressin increases the number of aquaporin 2 channels in the apical membrane and enhances the reabsorption of water in the kidney. The brain and nervous system are affected by pathological conditions in the kidney. Recent studies have shown that CKD and acute kidney injury adversely affect the structure and function of the brain and the nervous system [10–12]. However, there is still no consensus regarding the precise mechanism underlying the crosstalk between the kidney and the nervous system.
The neurological complications in patients with CKD are related to the peripheral nervous system and the CNS [13]. The prevalence of peripheral neuropathy is higher in patients with CKD than in the population with normal kidney function. Peripheral neuropathy includes myopathy, somatic neuropathy, and cranial neuropathy (olfactory, visual, and auditory nerves). CNS complications can be divided into cortical and subcortical disorders, and include cognitive disorders (encephalopathy, cognitive decline), vascular disorders (infarction, microbleeding, multifocal ischemia, posterior reversible encephalopathy), movement disorders (dystonia, chorea, tremor, reticular myoclonus), and sleep disorders (restless legs syndrome, sleep apnea) [10,13,14].
The severity of cognitive dysfunction varies from mild cognitive impairment to severe dementia, which can prevent patients from independently carrying out activities of daily living. Some CKD patients also suffer from cognitive dysfunction. The prevalence of depression is about three times higher in patients with CKD than the general population. Some studies have suggested that CKD patients have elevated risks of developing depression, dementia, and cognitive impairment [11,15,16]. In this review, we discuss the interactions between the kidney and the brain in CKD patients, focusing on the possible mechanisms that may contribute to neurological disorders in these patients.
ANXIETY AND DEPRESSION IN CKD
The prevalence rates of fatigue, depression, and anxiety in patients with chronic conditions are around 1.5 to 4 times higher than in the general population [12,17,18]. Patients with CKD have prevalence rates of anxiety disorders and anxiogenic symptoms of 19% and 43%, respectively [19]. Anxiety has a significant adverse effect on quality of life. Loosman et al. [20] reported that pre-dialysis CKD patients with elevated anxiety symptoms had 60% higher risks of mortality, hospitalization, and requiring dialysis than those without anxiety disorder. The most common risk factors of elevated anxiety symptoms are as follows: concomitant depression, reduced parathyroid hormone level, increased comorbidities, increased duration of hospitalization, decline in quality of life, and decreased vitality levels [19]. Persistently high or new-onset symptoms of anxiety are associated with reduced perceived social support in hemodialysis patients [21]. Regular screening for anxiety or depression as part of clinical care is therefore essential in patients with CKD and end-stage renal disease (ESRD). A number of anxiety screening tools and instruments, such as the Hospital Anxiety and Depression Scale (HADS), Beck Anxiety Inventory (BAI), and Generalized Anxiety Disorder 7 (GAD-7), have been used to evaluate the elevated anxiety symptoms of patients with CKD.
Depression is also common in the general population, with a lifetime risk of about 7% [22]. Chronic disease increases the risk of depression because it increases economic burden, reduces quality of life, and impairs activities of daily living. Very few randomized clinical trials of treatment have focused on screening CKD patients for depression. Moreover, the sample sizes were small, and the clinical outcomes were surrogates. The prevalence of depression in CKD patients was reported to be 26.5% based on screening questionnaires and 21.4% in clinical interviews with psychiatrists [23]. The risk factors of depression in CKD patients are young age, female sex, Black race, low education level, low family income, unemployment, hypertension, smoking status, diabetes, and coronary artery disease. A bidirectional association between depression and chronic disease has been identified [11]. The Diagnostic and Statistical Manual of Mental Disorders (DSM) includes the standard set of criteria used to diagnose mental disorders. Moreover, DSM has also been used to diagnosis depression in CKD patients [24]. For screening of depression in patients with CKD and ESRD, the most commonly used questionnaires that need to be validated are the Patient Health Questionnaire (PHQ-9), Beck Depression Inventory (BDI), Center for Epidemiologic Studies Depression Scale (CESD) 14, and Quick Inventory of Depressive Symptomatology Self-Report (QIDS-SR) [25–27].
MECHANISM OF DEPRESSION AND ANXIETY IN CKD
The prevalence of depression is high in CKD patients due to several factors, including uremic toxins, psychosocial symptoms, and economic challenges [8]. Although several mechanisms of depression have been reported in CKD patients, they could be broadly divided into behavioral and biological mechanisms. In this review, we focused on understanding the biological mechanisms.
Inflammation is frequently observed in CKD patients, which is related to adverse outcomes, including mortality [28,29]. Inflammation and depression have been suggested to show a bidirectional association in patients with chronic illness [30]. Other potential mechanisms include the development of comorbid cerebrovascular disease, which can also influence the incidence of depression in CKD patients. Moreover, cerebrovascular disease can increase the possibility of inflammation, which indirectly affects the mood of CKD patients. In clinical studies, it is difficult to establish a causal relationship between depression and CKD because many factors are involved. Experimental approaches can control for these factors, and help to understand the mechanism of depression in CKD patients. In a rodent CKD model, various behavioral tests were applied to evaluate sensorimotor function, mood, learning capacity, and memory. The results of these behavioral tests were validated and have been widely used in the literature describing how to evaluate neurological deficits in rodents (Table 1) [5,31–56].
The results of behavioral changes are disputed because of the diversity of CKD animal models, and the different time points for behavioral testing reflecting early or advanced stages of CKD [57–59]. Changes in the emotional behavior test were insufficient and ambiguous for CKD patients [9,60,61]. However, in our previous study in rats, we found that locomotor activity had declined in CKD rats [62]. Moreover, the time spent by the animals in the central zone of the open-field apparatus was reduced, indicating an increase in anxiety-related behaviors (Fig. 1) [62]. Many animal studies have shown that CKD itself causes changes in behavior, and leads to emotional and cognitive disorders (Table 2) [9,58,61–66].
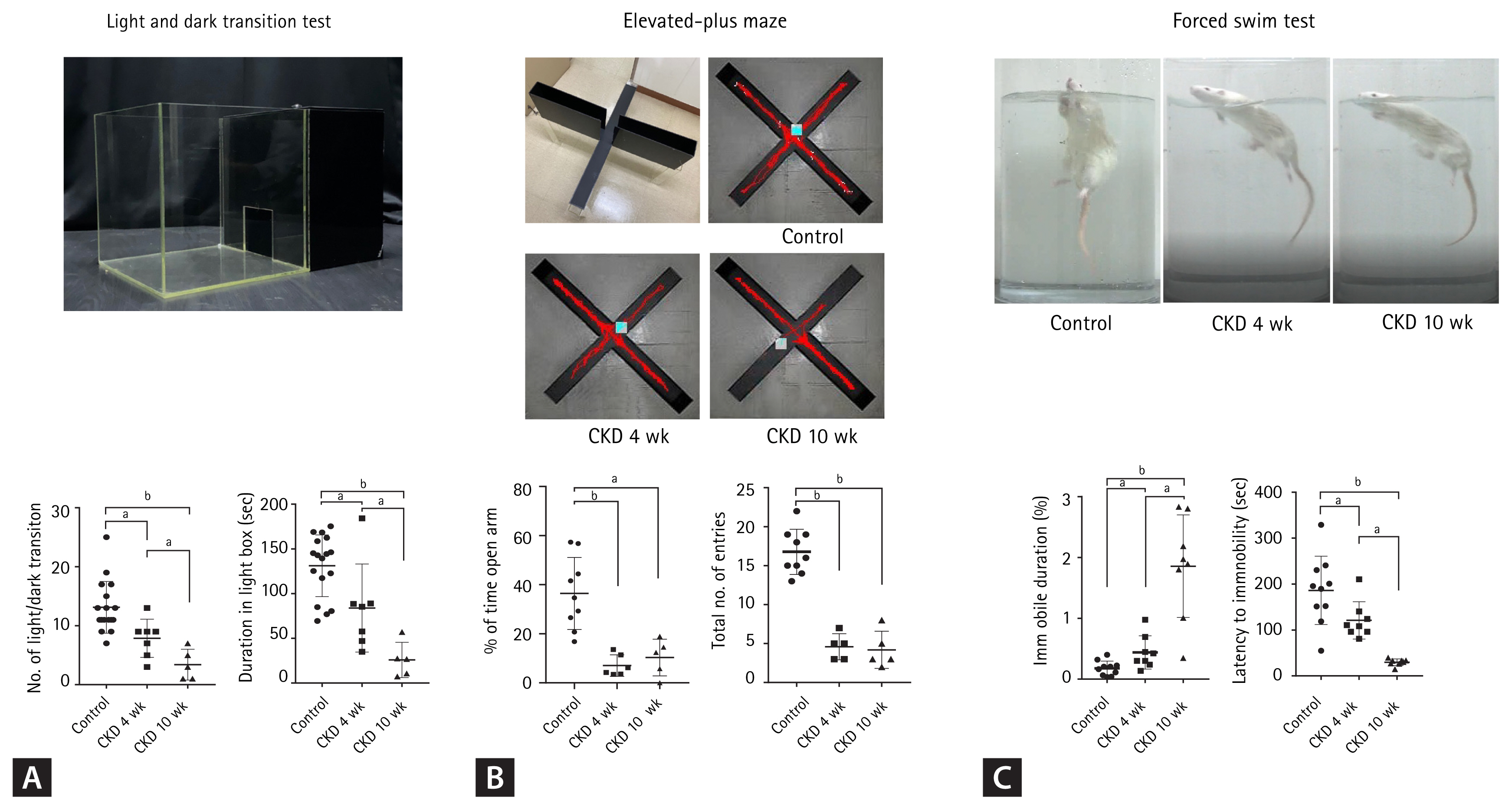
Behaviors of depression and anxiety in chronic kidney disease (CKD) rats. (A) The light-dark transition test apparatus consisted of a cage (30 × 30 × 30 cm) divided into two compartments by a black partition with a small opening that allowed the rat to move between the two compartments. One of the compartments was darkened, while the other was brightly illuminated. Rats were placed in the illuminated compartment and allowed to move freely for 5 minutes. The total time spent in the bright compartment was used as an indicator of anti-anxiety behavior. The light/dark transition number and the total time spent in the light compartment by CKD rats were lower than those of controls. (B) The elevated plus-maze test apparatus consisted of an elevated, plus-shaped (+) apparatus with two open arms and two enclosed arms. Rats were placed in an elevated (60 cm above the floor) plus maze that had two opposite open arms (50 × 10 cm each) and two opposite closed arms (50 × 10 cm each); the height of the walls was 50 cm. The number of entries into the maze and the time spent in individual arms were measured for 5 minutes. The percentage of entries into the open arms and the total number of entries in the elevated plus-maze were reduced for CKD rats compared to controls. (C) The forced swimming test was performed to measure depression-like behaviors. Rats were individually placed in a plastic cylinder (50 cm in height, 30 cm in diameter), which was filled with water (23°C ± 3°C). Their behavior was observed for 5 minutes, and their immobility time was calculated. The time spent immobile was taken as an indicator of depression-like behavior. During the forced swimming test, the percentages of immobility were higher for CKD rats than controls. A decreased latency to immobility was observed in CKD rats. Data are presented as the mean ± standard error of the mean. Modified from Yu et al. [62]. ap < 0.01, bp < 0.001, one-way analysis of variance.
Anxiety is described as the feeling of fear, uncertainty, helplessness, and apprehension that an individual encounters when anticipating a threatening situation [67]. In CKD, postulated mechanisms for increased susceptibility to anxiety may involve inflammatory processes secondary to uremic toxins, oxidative stress due to increased cytokine production, microvascular damage to the brain, and the involvement of the renin–angiotensin system [19]. However, this postulated mechanism of anxiety is similar to the development of depression or cognitive impairment in CKD. Further studies are needed, therefore, to elucidate specific mechanisms.
PREVALENCE OF COGNITIVE IMPAIRMENT IN CKD
CKD is one of the strongest risk factors for cognitive impairment. The current guidelines used in the diagnosis and management of mild cognitive impairment specify that kidney function should also be assessed as part of the routine neurological workup [5]. In fact, mild cognitive impairment is considered to be a prodromal state that initially occurs in patients with established dementia. The annual rate of progression from mild cognitive impairment to dementia is 1.9% in ESRD patients [16]. Mild cognitive impairment is more common among patients with stage 1–4 CKD (prevalence, 27% to 62%) than in matched populations without CKD (prevalence, 11% to 26%) [5,15,68–70]. The prevalence of mild cognitive impairment increases with the progression of CKD stage [71]. Dementia (Alzheimer’s disease, vascular dementia, Lewy body dementia, etc.) is not a specific disease but is, rather, a general term defined as an impaired ability to remember, think, or make decisions that interfere with everyday activities. In the general population, age is a very important risk factor for dementia because the incidence of dementia is very low (< 1%) in individuals < 65 years old [72]. However, in young patients with ESRD, the prevalence of cognitive impairment may be higher than that in the general population [73]. Another study showed that the overall age- and sex-specific incidence rates and the cumulative incidence rates of dementia were much higher in ESRD than non-ESRD subjects (10.73 vs. 1.40 per 1,000 person-years and 0.061 vs. 0.017, respectively, both p < 0.0001) [74]. Age-related chronic diseases, such as cardiovascular disease, high cholesterol, diabetes, and kidney disease, are factors that cause the advancement and rapid progression of dementia [75]. Therefore, CKD itself could be related to the progression and prevalence of dementia.
PATTERN OF COGNITIVE IMPAIRMENT IN CKD
Cognitive impairment is assessed by analyzing specific cognitive domains, such as attention, memory, visuospatial ability, language skills, and execution skills [71]. Recent studies have suggested that the phenotype of cognitive dysfunction in patients with CKD may be different from that in the general population [71]. Attention is defined as the selection of specific information within a sensory channel, i.e., filtering the essential data and ignoring the remaining data. The prefrontal cortex performs this activity, which is modulated by the dopaminergic system. Lizio et al [76]. directly compared CKD-associated dementia with Alzheimer’s disease, and they found a more pronounced dysfunction in the frontal cortex of patients with CKD and mild cognitive impairment compared to the general population. The memory storage capacity and the processing of information are impaired in patients with CKD [77]. Some animal studies demonstrated neuronal death in the hippocampus associated with CKD [62,78,79]. In addition, CKD was shown to reduce the activity of cholinergic neurons in the nucleus basalis of Meynert [80]. Abnormal interactions occur between these cholinergic neurons and the cortical neurons, causing memory dysfunction in CKD. Griva et al. [81] reported a statistically significant improvement in the memory performance of patients following kidney transplantation. Language skills are also affected in patients with CKD, and this is the only cognitive domain that shows a linear relationship with declining estimated glomerular filtration rate (eGFR) [24]. Although magnetic resonance imaging (MRI) studies did not show any anatomical changes in the cortical language areas [82], poor naming performance was observed in patients with CKD [83,84]. Several studies have shown that executive dysfunction occurs in patients with CKD measured by the Trail Making Test type A (TMT-A) and the TMT-B, which test cognitive processing speed [76,83,85,86]. Executive dysfunction worsens with a decline in the eGFR [86]. Executive functions are mainly carried out by the frontal lobe, which is thinner in patients with CKD. Cognitive impairment of patients with CKD occurs due to dysregulation of several functional domains of the brain. In fact, it may occur due to the damage caused to multiple cortical regions (particularly the frontal lobe) and subcortical modulatory neurons, especially the adrenergic neurons in the mesencephalon and the cholinergic neurons in the nucleus basalis of Meynert [24]. However, non-CKD dementia is caused by structural abnormalities in different regions of the brain, as revealed by MRI analyses. The differences in the structural brain changes between patients with CKD and non-CKD dementia may be attributed to the different mechanisms of cognitive dysfunction that occur in patients with CKD.
MECHANISM OF COGNITIVE IMPAIRMENT IN CKD
The mechanism of cognitive impairment in CKD is not well understood. Genetic factors lead to the pathogenesis of cognitive dysfunction in pediatric patients with CKD [71,87]. However, vascular injury and uremic toxins are suspected to induce cognitive impairment in adult patients with CKD [10]. Atherosclerosis and endothelial dysfunction are common conditions in patients with CKD. The kidney and the brain have “strain vessels,” i.e., afferent arterioles in the kidney and perforating arterioles in the brain. These vessels are short in length, because small arterioles branch out of very large arteries that autoregulate tissue perfusion [88]. The anatomical vascular systems are similar in both of these organs, which may be susceptible to the traditional risk factors, i.e., hypertension, diabetes, hyperlipidemia, and obesity [14]. As many risk factors are involved in the development of CKD and vascular dysfunction, vascular factors could also be involved in the development of cognitive impairment in patients with CKD. In the general population, reduced cerebral blood flow impairs neural activity, which is involved in the pathogenesis of cognitive impairment. However, the global cerebral blood flow was found to be higher in CKD patients than in healthy individuals, which does not support the more general notion of vascular dysfunction in patients with CKD [89]. Prior to peritoneal dialysis, ESRD patients were shown to have cerebral hyperperfusion with reduced execution function [90]. Increased cerebral blood flow probably occurs due to anemia in patients with CKD [90,91]. This discrepancy makes it difficult to apply directly the mechanism of cognitive impairment determined in the general population to patients with CKD.
In patients with CKD, vascular injury can lead to white matter lesions, silent infarction, and cerebral microbleeds. Leukoaraiosis is a pathological appearance of the brain white matter, which is thought to be due to perfusion abnormalities within the arterioles that perforate through the deep brain structures. Leukoaraiosis is thought to represent ischemia and is associated with increased risk of stroke and dementia [92,93]. The prevalence of leukoaraiosis is higher in patients with CKD than in the general population [94].
Uremic toxins accumulate in the body fluids of patients with progressive CKD, and have a direct impact on the development of cerebrovascular disease. Recent studies have reported that uremic toxins cause cognitive impairment in patients with CKD. A recent review article established that uremic toxins, such as uric acid, indoxyl sulfate, p-cresyl sulfate, interleukin-1β (IL-1β), IL-6, tumor necrosis factor-α, and parathyroid hormone, are likely to have an impact on the CNS, although the underlying mechanisms have yet to be elucidated [95]. In addition, guanidine compounds, asymmetric dimethylarginine, fibroblast growth factor 23, excessive phosphate, and indoxyl sulfate have also been considered potential uremic neurotoxins.
Indoxyl sulfate is a protein-bound uremic toxin, which is also known to be a renal and vascular toxin as it causes nephrotoxicity, especially in tubular cells, and inhibits the proliferation of endothelial cells; thus, indoxyl sulfate induces free radicals [96,97]. In endothelial cells, aryl hydrocarbon receptor (AhR) may be the receptor of indoxyl sulfate. When indoxyl sulfate activates AhR, it causes endothelial dysfunction by inducing endothelial inflammation and an increase in oxidative stress. This leads to the development of a procoagulant state. Moreover, AhR is widely expressed in the CNS, and activated AhR was shown to have detrimental effects on cognitive functions. Recent studies suggested that indoxyl sulfate induces neurotoxicity via the proposed mechanism shown in Fig. 2. Adesso et al. [98] reported that indoxyl sulfate causes inflammation and oxidative stress in primary cells of the CNS by activating nuclear factor-κB (NF-κB) and AhRs, resulting in the induction of neuronal death. Indoxyl sulfate plays a role in apoptosis via inhibition of the mitogen-activated protein kinase (MAPK) pathway in human astrocytes under conditions of oxidative stress [99]. Bobot et al. [63] reported that rats fed an adenine-rich diet with drinking water containing indoxyl sulfate showed an increased serum concentration of indoxyl sulfate and stronger impairment of cognition and increased permeability of the blood-brain barrier (BBB). Consequently, uremic toxins accumulated in the brain as the BBB was disrupted due to increased indoxyl sulfate concentration. The glymphatic system is a waste clearance system in the CNS, which is formed by astroglial cells that efficiently eliminate soluble proteins and various metabolites from the CNS. Intriguingly, the glymphatic system functions mainly during sleep, and is largely disengaged during wakefulness. Sleep disturbances are common in patients with CKD. Further studies are required to determine how uremic toxins and/or CKD influence the glymphatic system, which can suppress the clearance of uremic toxins and β-amyloid.
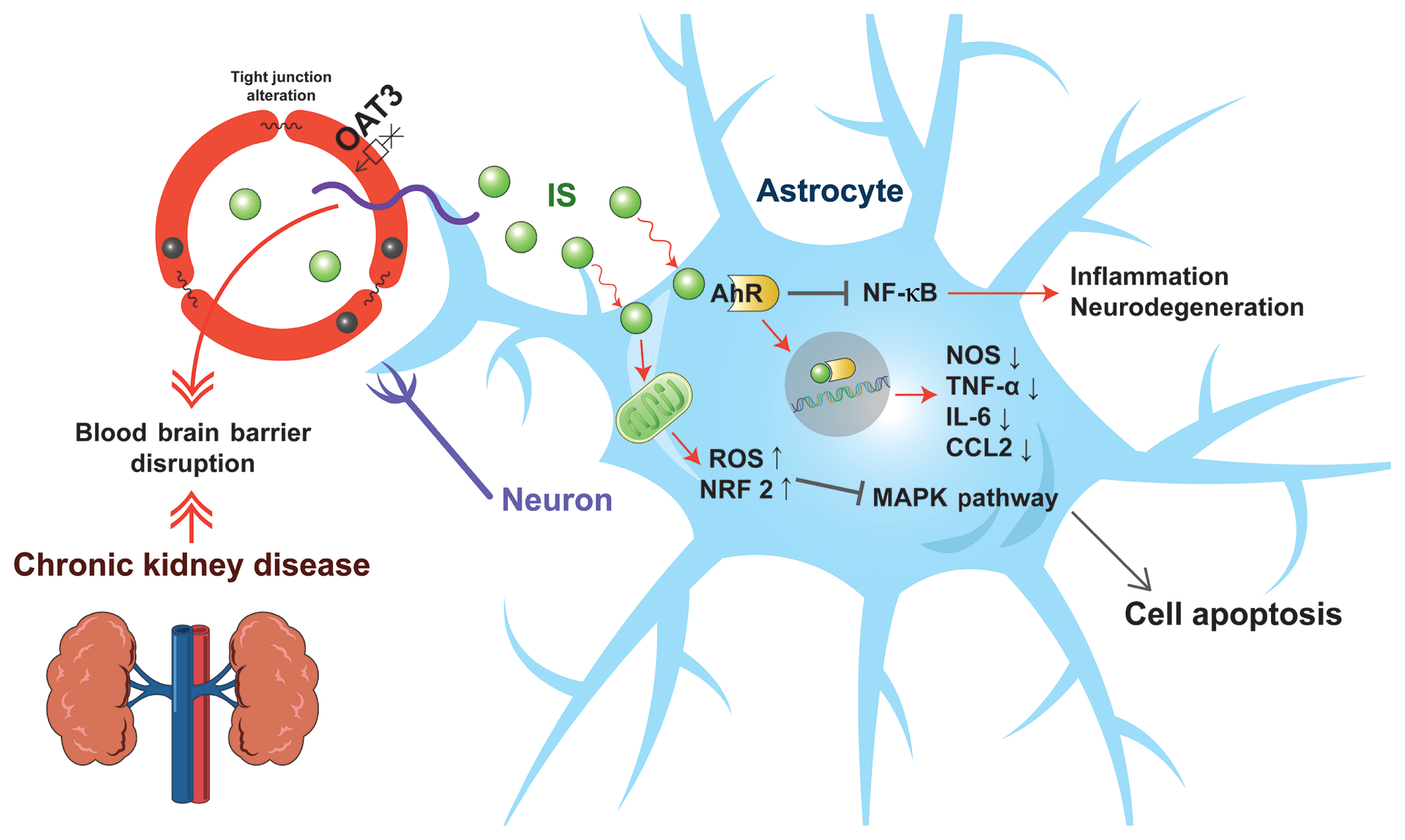
Proposed scheme of indoxyl sulfate-induced neurotoxicity. Indoxyl sulfate disrupts the integrity of the blood-brain barrier. In addition, indoxyl sulfate inhibits organic anion transporter 3 (OAT3) and efflux transport of endothelial cells, resulting in the accumulation of indoxyl sulfate in the brain. Furthermore, indoxyl sulfate increases reactive oxygen species (ROS) in astrocytes, inhibiting the mitogen-activated protein kinase (MAPK) pathway and inducing apoptosis. Moreover, indoxyl sulfate binds with aryl hydrocarbon receptor (AhR), and activates the signaling of AhR in astrocytes. The activated AhR inhibits the transcription of nitric oxide synthase (NOS), tumor necrosis factor-α (TNF-α), interleukin 6 (IL-6), and C-C motif chemokine ligand 2 (CCL2), which show antiinflammatory effects. Finally, indoxyl sulfate suppresses nuclear factor-κB (NF-κB) signals in astrocytes, which promote inflammation and neurodegeneration in the CNS. NRF2, nuclear factor erythroid 2-related factor 2.
CONCLUSIONS
Clinical studies have consistently established that there is a relationship between CKD and emotional/cognitive changes. Genetic factors, inflammation, cerebrovascular disease, vascular factors, and uremic toxins may be associated with the development and progression of cognitive changes in patients with CKD. Radiological studies have elucidated the different mechanisms of CKD dementia and compared them with the characteristics of non-CKD dementia. Furthermore, animal studies have established the mechanism underlying the brain damage caused by CKD. However, discrepancies occurred between behavioral tests, and further studies are required to determine the detailed mechanisms. Finally, research should also focus on identification of potential therapeutic targets to prevent changes in the brains of patients with CKD.
Notes
This manuscript was contributed by The Korean Society of Nephrology.
No potential conflict of interest relevant to this article was reported.
Acknowledgements
This work was supported by the Basic Science Research Program (2021R1F1A1060352 and 2017R1D1A1B05036195) through the National Research Foundation of Korea funded by the Ministry of Education and the Soonchunhyang University Research Fund.