Effects of aging on accompanying intermittent hypoxia in a bleomycin-induced pulmonary fibrosis mouse model
Article information
Abstract
Background/Aims
Obstructive sleep apnea (OSA) is prevalent in older patients with idiopathic pulmonary fibrosis (IPF); however, it is underrecognized. OSA is characterized by intermittent hypoxia (IH) and sleep fragmentation. In this study, we evaluated the effects of IH in an older mouse model of bleomycin-induced lung fibrosis.
Methods
Bleomycin-induced mice (C57BL/6, female) were randomly divided into four groups of young vs. old and room air (RA)-exposed vs. IH-exposed. Mice were exposed to RA or IH (20 cycles/h, FiO2 nadir 7 ± 0.5%, 8 h/day) for four weeks. The mice were sacrificed on day 28, and blood, bronchoalveolar lavage (BAL) fluid, and lung tissue samples were obtained.
Results
The bleomycin-induced IH-exposed (EBI) older group showed more severe inflammation, fibrosis, and oxidative stress than the other groups. The levels of inflammatory cytokines in the serum and BAL fluid increased in the EBI group. Hydroxyproline levels in the lung tissue increased markedly in the EBI group.
Conclusions
This study demonstrates the possible harmful impact of OSA in an elderly mouse model of lung fibrosis. This study further suggests that older patients with IPF and OSA may be more of a concern than younger patients with IPF. Further research is required in this area.
INTRODUCTION
Idiopathic pulmonary fibrosis (IPF) is a fatal chronic disease that causes respiratory failure and has a median survival of 2–3 years from the time of diagnosis [1]. IPF progression in older adults is characterized by the restriction of pulmonary function due to fibrosis of the interstitial area [1]. Common comorbidities are cardiovascular disease, pulmonary hypertension, gastroesophageal reflux disease (GERD), and obstructive sleep apnea (OSA) [2]. Among these, OSA is particularly frequent (prevalence 6–91%) and is closely associated with the quality of life and prognosis in patients with IPF [3-6].
OSA is characterized by intermittent hypoxia (IH), a condition in which periods of normoxia alternate with hypoxia and sleep fragmentation [7]. Moreover, chronic hypoxia is frequent in IPF and promotes systemic inflammation and pulmonary vascular damage [8,9]. Various studies have suggested that if IH, a key mechanism underlying OSA, co-occurs with IPF, it may cause greater oxidative stress and systemic inflammation than IPF alone [10,11]. Recent clinical and experimental data have shown that IH is closely associated with disease progression and poor outcome in patients with IPF. However, IH has not been adequately studied in aging population with IPF.
In this study, we investigated the effects of IH on a bleomycin-induced pulmonary fibrosis using mouse models, aged 8 weeks (young) and 24 months (older), to characterize alterations in inflammation and pulmonary structures.
METHODS
Mice and experimental groups
All experiments were performed using young (eight wk old) and old (24 mo old) mice. Pathogen-free female C57BL/6 mice were obtained from the Korea Research Institute of Bioscience and Biotechnology (KRIBB). Mice were maintained on a 12-h light, 12-h dark cycle with constant humidity, temperature, and free access to water and rodent feed in individual cages.
The experimental design is illustrated in Figure 1. Mice were randomly allocated to four groups (six to eight mice per group): young + bleomycin + room air (YBC), young + bleomycin + IH (YBI), old + bleomycin + room air (EBC), and old + bleomycin + IH (EBI). After 0.01 U bleomycin (Sigma, St. Louis, MO, USA) was intratracheally instilled, the mice were placed in the Oxycycler chambers for four weeks and exposed to IH (approximately 7 ± 0.5% fractional inspired O2 (FiO2), 20 episodes/h, 8 h during daytime; to coincide with mouse sleep cycles) or room air. The mice were sacrificed at four weeks. The control mice were treated with equal volumes of saline. Oxygen levels were monitored with a ProOx 110 analyzer, and air, nitrogen, and oxygen flow flowing into the cage were regulated using BioSpherix computer software (BioSpherix Oxycycler, Redfield, NY, USA). Mice exposed to room air and oxygen were simultaneously evaluated and their body weights were measured weekly. This study was approved by the Ethical Committee on Animal Experiments of the Catholic University of Korea (approval number EPSMH20203003FA) and complied with the animal welfare guidelines.
Analysis of bronchoalveolar lavage fluid (BALF)
BALF samples were harvested through the trachea into the lung using a 23-gauge catheter and lavaged with 800 μL of Dulbecco's phosphate-buffered saline (DPBS). BALF was centrifuged at 1,500 rpm for 5 minutes at 4°C. Differentiated cells were centrifuged (5 min at 750 g) on glass slides and stained with Diff-Quick (Sysmax, Tokyo, Japan). Total BAL cells were counted using a hemocytometer, and the types of inflammatory cells (macrophages, neutrophils, eosinophils, and lymphocytes) were counted in at least 400 cells in randomly selected areas of the slide.
Histopathologic evaluation
For histopathological examination, the left lung tissues from the mice in each group were fixed overnight in a 4% para-formaldehyde solution and processed for paraffin embedding. Sections were cut to a thickness of 3 μm using a microtome and stained with hematoxylin and eosin (H&E) and Masson’s trichrome for histological examination. Images were acquired using a Panoramic MIDI slide scanner (3DHISTECH Ltd., Budapest, Hungary), and each stained slide image was randomly screened. The Szapiel score was used to evaluate the degree of the parenchymal alveolitis [12]. The Ashcroft scale was used to evaluate fibrotic changes [13]. Five randomly chosen fields within each lung section were observed (×100 or ×200 magnification), and two independent blind observers scored each specimen. The mean Szapiel or Ashcroft score for each mouse was used for the statistical analysis.
Lung wet-dry weight ratio
The middle lobe of the right lung portion was immediately weighed after collection (wet weight), placed into a 65°C oven for 48 hours, and weighed again (dry weight). The wet-dry lung tissue ratio was calculated by dividing the wet weight by the dry weight. The ratio of wet and dry lung weights is a measure of edema formation and a marker of acute lung injury.
Inflammatory cytokine assay
The Cytometric Bead Array (CBA; BD Bioscience, Franklin Lakes, NJ, USA) kit, a flow cytometry application that allows users to quantify multiple proteins simultaneously, was used to analyze inflammatory cytokines. The kit was designed to detect the cytokines interleukin (IL)-12p70, tumor necrosis factor (TNF), interferon (IFN)-γ, monocyte chemoattractant protein (MCP)-1, IL-10, and IL-6 in BAL The analysis was performed according to the manufacturer’s instructions.
Collagen concentration assay
Hydroxyproline content of the lungs is considered a quantitative biochemical measure of collagen deposition. Hydroxyproline levels in the lungs were examined using a commercial kit (BioVision, Inc., Milpitas, CA, USA) according to the manufacturer’s instructions. Lung homogenates were briefly hydrolyzed with 12 N hydrochloric acid for 12 hours at 110–120°C. Chloramine T solution and DMAB reagent were added to the acid extract and incubated for 90 minutes at 60°C. Transforming growth factor beta (TGF-β) level was determined using a commercial TGF-β enzyme-linked immunosorbent assay (ELISA) Kit (Thermo Fisher Scientific, Inc., Waltham, MA, USA), according to the manufacturer’s instructions. The absorbances of the samples were measured at 560 nm and 450 nm.
Oxidative stress assay
An indicator of polymorphonuclear leukocyte accumulation, myeloperoxidase (MPO; BioVision, Inc.), albumin, lipid peroxidation enzyme malondialdehyde (MDA; Abcam, Burlingame, CA, USA), oxidative stress-related enzyme catalase (CAT), superoxide dismutase (SOD), and glutathione (GSH) (Bioassay Systems, Hayward, CA, USA) in BALF or serum were measured using commercial mouse ELISA kits as according to the manufacturer’s instructions. Chemiluminescence was detected using an ELISA plate reader. All samples and standards were analyzed in duplicate.
Isolation of primary fibroblast cells and senescence-associated β-galactosidase (SA-β-gal) staining
Lung tissues were obtained from euthanized mice and washed with DPBS to remove blood. The tissue was cut into small pieces using an autoclaved surgical blade and scissors and placed in a cell culture hood. Cells were dissociated by adding 5 mL medium (Dulbecco’s modified eagle’s medium high glucose + 1% penicillin/streptomycin + 1 mg/mL collagenase A) into the minced tissue in a conical tube, placing the sample tubes horizontally on a shaker, and gently shaking them at 37°C for 4 hours. After incubation, each sample was filtered through a 70 µm cell strainer. The cells were centrifuged at 1,300 rpm for 3 minutes using a refrigerated centrifuge. The supernatant was removed, and the pellets were resuspended in 5 mL minimal medium (without fetal bovine serum). The fibroblasts were maintained at 37°C in a humidified 5% CO2 incubator and seeded 48 hours prior to staining at 5 × 104 cells/well in 6-well plates. Cells were stained with SA-β-gal and analyzed using a Cellular Senescence Assay Kit (CBA-230; Cell Biolabs Inc., San Diego, CA, USA) according to the manufacturer’s protocol.
Quantitative reverse-transcription polymerase chain reaction (qRT-PCR)
Total RNA from the lung tissue was extracted using TRIzol (Invitrogen, Carlsbad, CA, USA), and qRT-PCR was performed using the QuantiFast SYBR Green PCR kit (Qiagen, Valencia, CA, USA) to analyze the changes in the mRNA expression of the senescence markers, p21 and p53. The primer sequences were designed based on the NCBI database. The threshold cycle (Ct) value was normalized to that of β-actin. The relative expression levels were determined by the 2-ΔΔCt method.
Western blot analysis
Total protein was obtained from the homogenized lung tissue, and equal amounts of protein were separated by 10–15% sodium dodecyl sulfate polyacrylamide gel elctrophoresis and transferred to polyvinylidene fluoride membranes. The membranes were blocked and incubated at 4°C overnight with the primary antibodies p53 and-actin—as controls (Cell Signaling Technology, Danvers, MA, USA). Target proteins were detected using an Image Quant LAS 500 (GE Healthcare Bio-Sciences AB, Uppsala, Sweden).
Terminal deoxynucleotidyl transferase dUTP nick end labeled (TUNEL) assay
Apoptotic cells in lung tissue sections were detected with the TUNEL Assay Kit using an in situ apoptosis detection kit (Promega, Madison, WI, USA), according to the manufacturer’s protocol. The TUNEL-positive cells (dark brown-stained nuclei) were semi-quantitatively assessed using a light microscopy in ten random areas of lung sections under the power field of microscope (400 × magnification).
Immunohistochemistry (IHC)
For IHC staining, antigen retrieval was performed by incubation in a citrate buffer (pH 6.0) and boiling in a microwave oven for 15 minutes. After blockage of endogenous peroxidases was performed, slides were incubated with anti-p21 antibodies at 4°C overnight and subsequently incubated with biotin-labeled secondary antibodies. Immunoreactive signal was visualized with 3,3′-Diaminobenzidine substrate kit (Vector Laboratories, Burlingame, CA, USA), and counter-staining was performed with hematoxylin.
Statistical analysis
Statistical analyses were performed using the GraphPad Prism software, version 7.00 (GraphPad Software Inc., San Diego, CA, USA). The test data were analyzed using one-way analysis of variance (ANOVA), followed by Tukey’s multiple comparison test or two-way ANOVA (for BAL cell differentiation). Data are expressed as the mean ± standard deviation, and p < 0.05 was considered statistically significant.
RESULTS
Effects of bleomycin and IH on lung injury, inflammation and oxidative stress
Histopathological analysis showed more severe lung injury with inflammatory cell infiltration and thickening of alveolar septa in the older mice groups, with the EBI group having more severe lung injury and inflammation than the other groups (Fig. 2A). Total cell and lymphocyte counts in the BALF were significantly higher in the EBI group than in the other groups (all p < 0.001) (Fig. 2D). The lung wet/dry ratio and albumin levels, which are markers of lung injury, significantly increased in the older mice groups, with the EBI group showing the largest increase (Fig. 2B, C). Szapiel score was significantly increased in the EBI group compared to other groups (Fig. 2E). MPO activity in lung homogenates and MCP-1 in BALF significantly increased in the EBI group compared to the other groups (Fig. 3A, E). The TNF, INF-γ, IL-10, and IL-6 levels in BALF showed increasing trends in the EBI group compared to other groups (Fig. 3C, D, F, G).
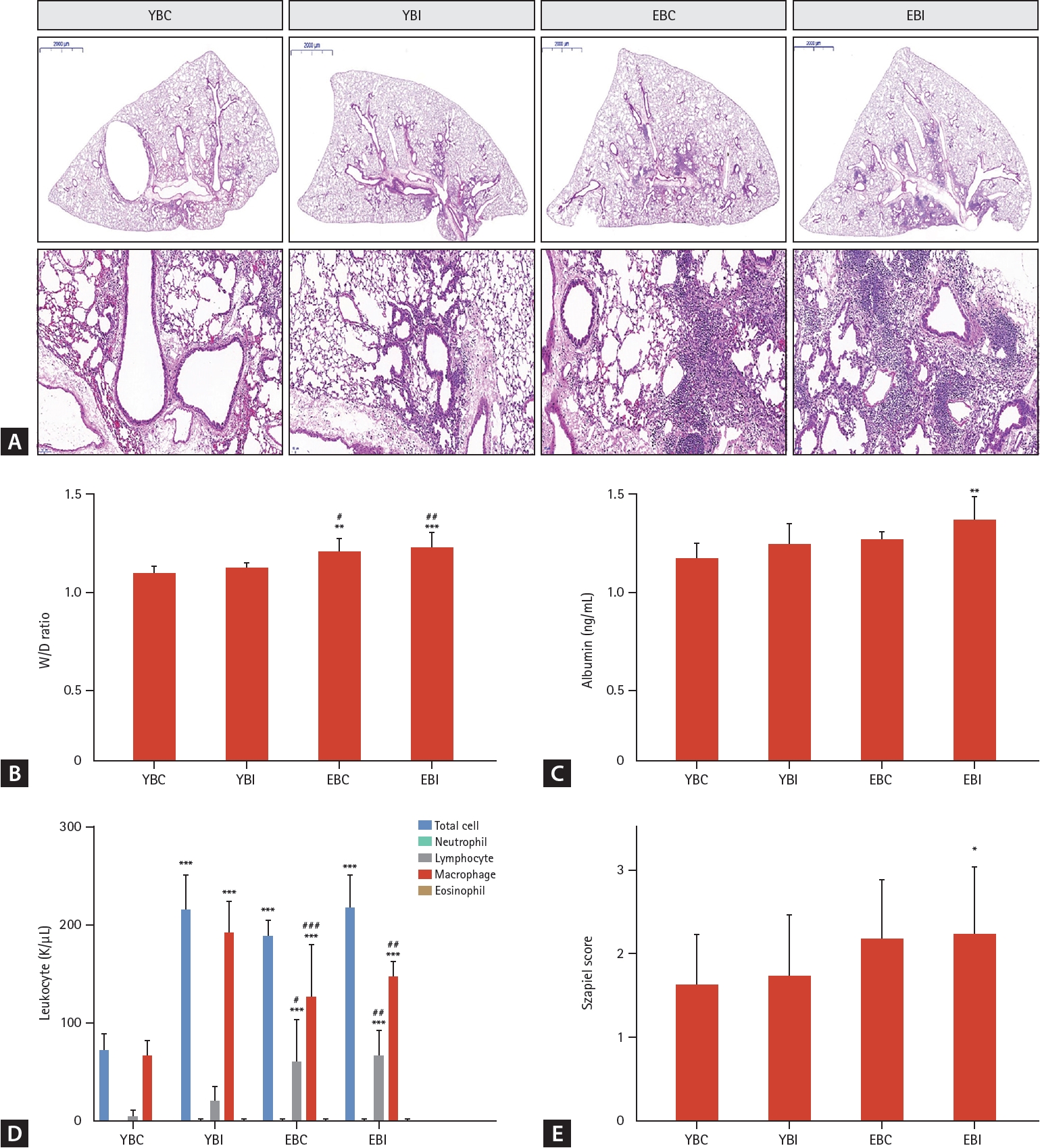
Histopathologic analysis of the effects of bleomycin and IH on lung injury and inflammation. (A) Representative photomicrographs of lung tissues were stained with H&E. The panel shows an image of the whole lung section (scale bars: 2,000 μm). The images are enlarged in the lower panels (magnification, × 200). (B) Lung W/D ratio and (C) albumin were analyzed as markers of lung injury. (D) BAL cells were counted to analyze the numbers of inflammatory cells. (E) Szapiel’s score was used to evaluate alveolar inflammation and lung injury. Data represent the mean ± standard deviation. BAL, bronchoalveolar lavage; EBC, old + bleomycin + room air; EBI, old + bleomycin + intermittent hypoxia; H&E, hematoxylin and eosin; IH, intermittent hypoxia; W/D, wet/dry; YBC, young + bleomycin + room air; YBI, young + bleomycin + intermittent hypoxia. *p < 0.05, **p < 0.01, ***p < 0.001 vs. YBC; #p < 0.05, ##p < 0.01, ###p < 0.001 vs. YBI.
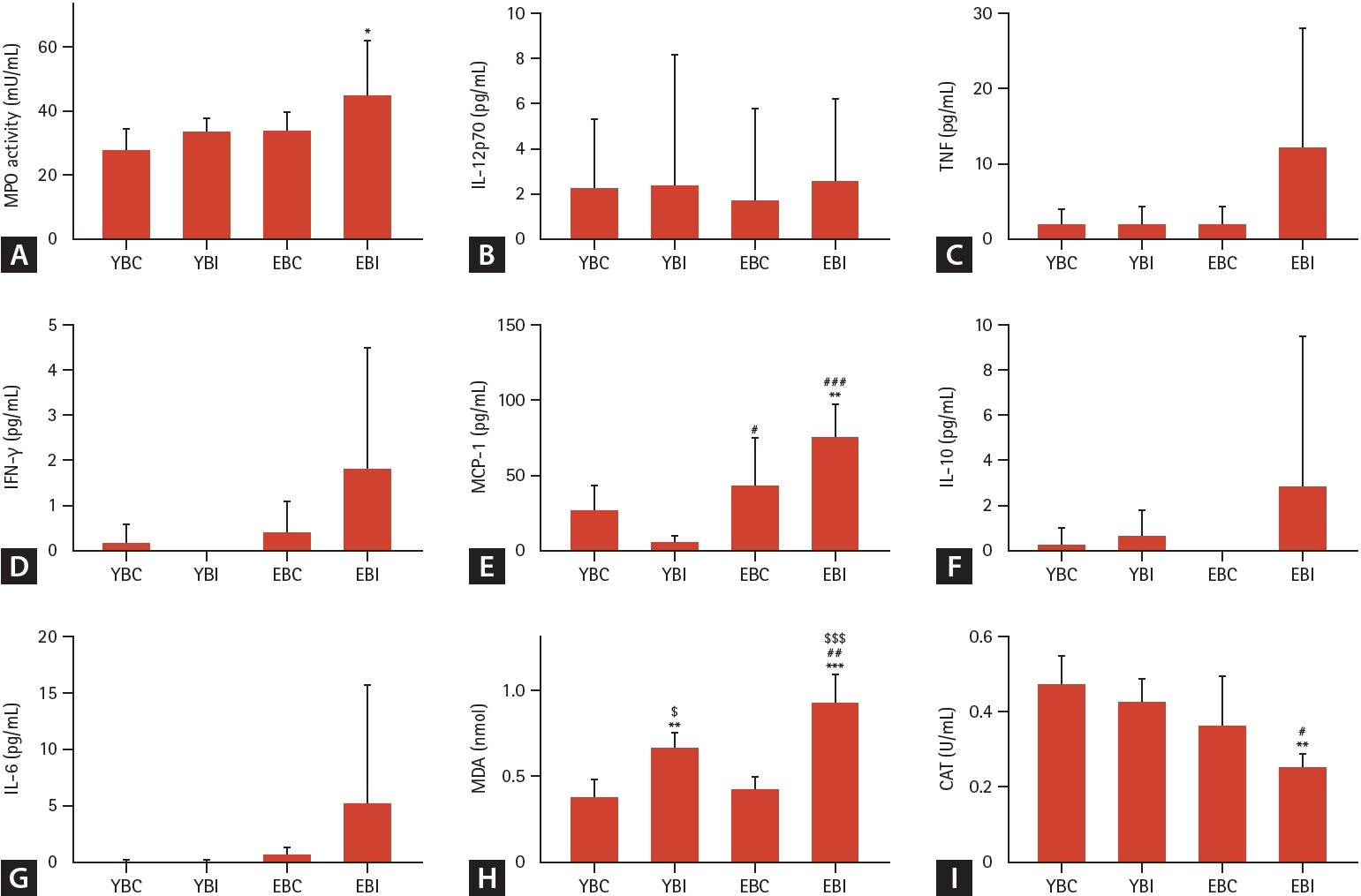
Effects of bleomycin and intermittent hypoxia on inflammation and oxidative stress in young and elderly mice. (A) MPO as an inflammatory enzyme was measured in total lung homogenates. (B-G) Cytometric Bead Array; IL-12p70, TNF, IFN-γ, MCP-1, IL-10, and IL-6 in BAL fluid were measured as inflammatory cytokines. The levels of (H) MDA, and (I) CAT activity were measured as indicators of oxidative stress in total lung homogenate. Data represent the mean ± standard deviation. BAL, bronchoalveolar lavage; CAT, catalase; EBC, old + bleomycin + room air; EBI, old + bleomycin + intermittent hypoxia; IFN, Interferon; IL, interleukin; MCP, monocyte chemoattracted protein; MDA, malondialdehyde; MPO, myeloperoxidase; TNF, tumor necrosis factor; YBC, young + bleomycin + room air; YBI, young + bleomycin + intermittent hypoxia. *p < 0.05, **p < 0.01, ***p < 0.001 vs. YBC; #p < 0.05, ##p < 0.01, ###p < 0.001 vs. YBI; $p < 0.05, $$$p < 0.001 vs. EBC.
The levels of MDA, CAT, SOD, and GSH were measured as indicators of oxidative stress in the total lung homogenate. The MDA levels in the lungs were significantly higher in the EBI group than in the other groups (Fig. 3H). The CAT levels were significantly lower in the EBI group than in the other groups (Fig. 3I). SOD activity and GSH levels did not differ significantly among the four groups (data not shown).
Effects of bleomycin and IH on lung fibrosis
Masson’s trichrome staining of the lung tissues demonstrated more severe lung injury, including inflammatory cell infiltration and thickening of the alveolar septa, in the EBI group than in the other groups (Fig. 4A). The hydroxyproline count in the lung tissue and the TGF-ß level in BALF significantly increased in the older groups, especially the EBI group, compared to the other groups (p < 0.001) (Fig. 4B, C).
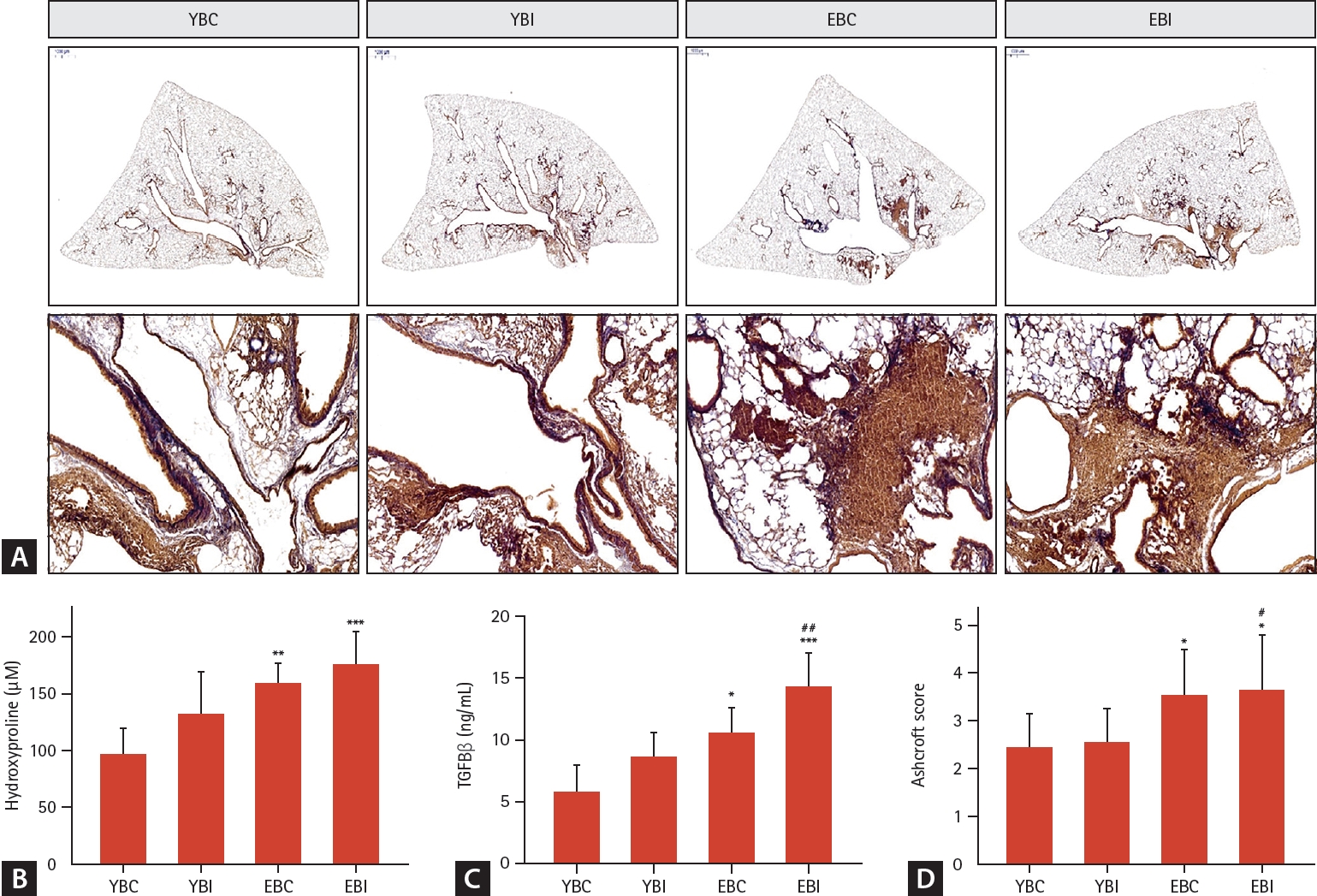
Effects of bleomycin and intermittent hypoxia on lung fibrosis in young and elderly mice. (A) Representative photomicrographs of lung tissues stained with Masson’s trichrome to determine collagen accumulation (scale bars: 2,000 μm). The images are enlarged in the lower panels (magnification, × 200). (B) Hydroxyproline content was measured in lung tissue. (C) TGF-β was measured in BAL fluid by ELISA. (D) Ashcroft score, and collagen deposition were analyzed. Data represents the mean ± standard deviation. BAL, bronchoalveolar lavage; EBC, old + bleomycin + room air; EBI, old + bleomycin + intermittent hypoxia; TGF, transforming growth factor; YBC, young + bleomycin + room air; YBI, young + bleomycin + intermittent hypoxia. *p < 0.05, **p < 0.01, ***p < 0.001 vs. YBC; #p < 0.05, ##p < 0.01 vs. YBI.
Effects of bleomycin and IH on senescence
After isolation of primary fibroblasts in lung, SA-β-gal staining increased significantly in the elderly groups, especially the EBI group (Fig. 5A).
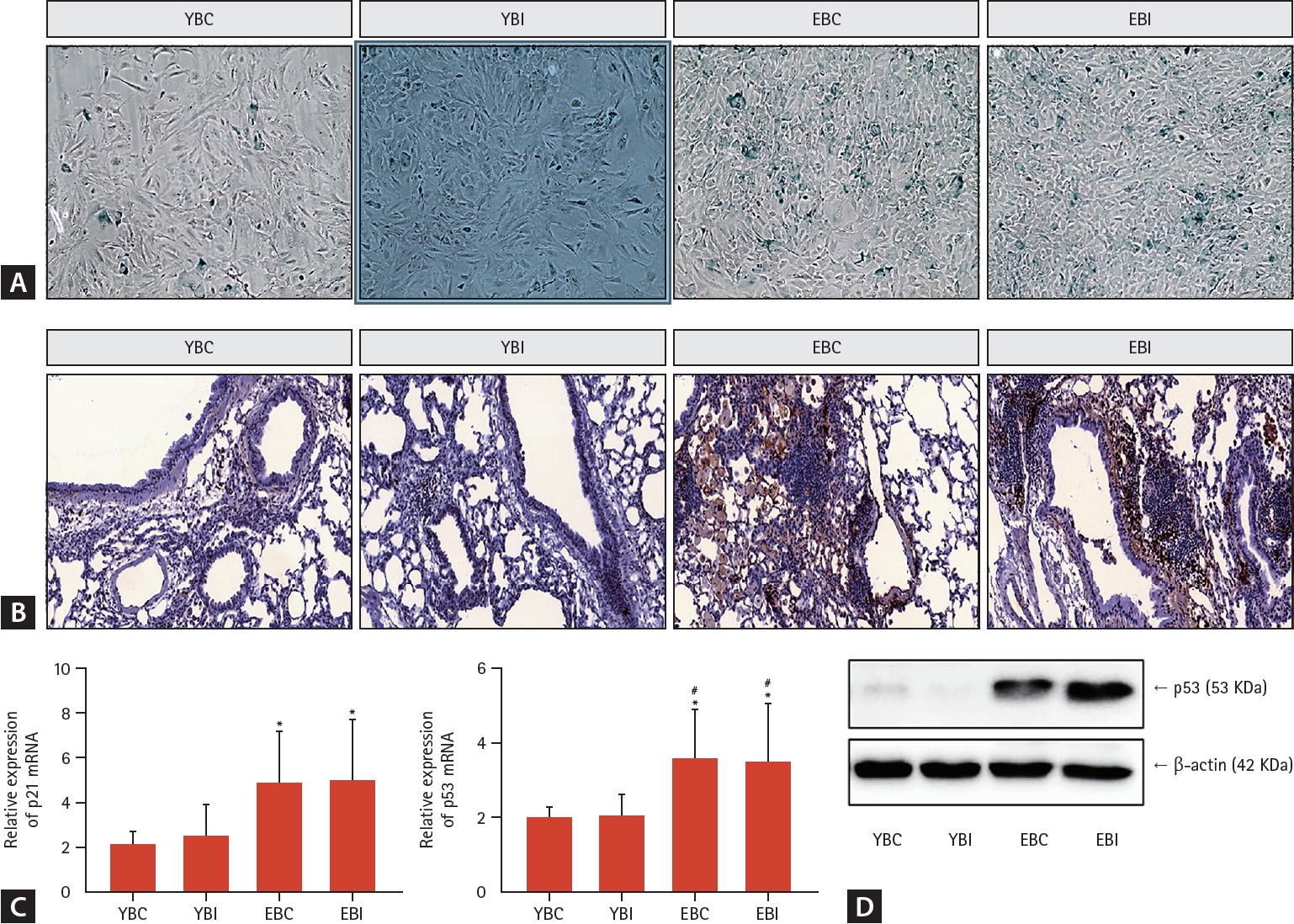
Effects of bleomycin and intermittent hypoxia on senescence in young and elderly mice. (A) After isolation of primary fibroblasts in lung, SA-β-gal staining was performed. (B) Immunohistochemistry was performed on lung tissues with p21 antibody (magnification, × 200). (C) mRNA expression of p21 and p53 as the senescence markers was examined by qRT-PCR. (D) Protein levels of p53 was examined in total protein by western blotting. β-actin was used as a normalization gene. Data represent the mean ± standard deviation. EBC, old + bleomycin + room air; EBI, old + bleomycin + intermittent hypoxia; SA-β-gal, senescence-associated β-galactosidase; YBC, young + bleomycin + room air; YBI, young + bleomycin + intermittent hypoxia. *p < 0.05 vs. YBC; #p < 0.05 vs. YBI.
While the younger groups had no immunoreactive cells, tissue sections from the elderly groups, especially the EBI group, were immunoreactive for p21 (Fig. 5B). The elderly groups, especially the EBI group, had significantly higher levels of p21 and p53 mRNA compared to the younger groups (Fig. 5C). Immunoblotting for p53 showed higher levels of this protein in the lung tissues from older mice than those from the younger groups (Fig. 5D).
Effects of bleomycin and IH on apoptosiss
TUNEL-positive cells (apoptotic nuclei, Fig. 6A) were more evident in the elderly mice groups than in younger mice groups. The combination of bleomycin and IH significantly increased the number of TUNEL-positive cells (Fig. 6B).
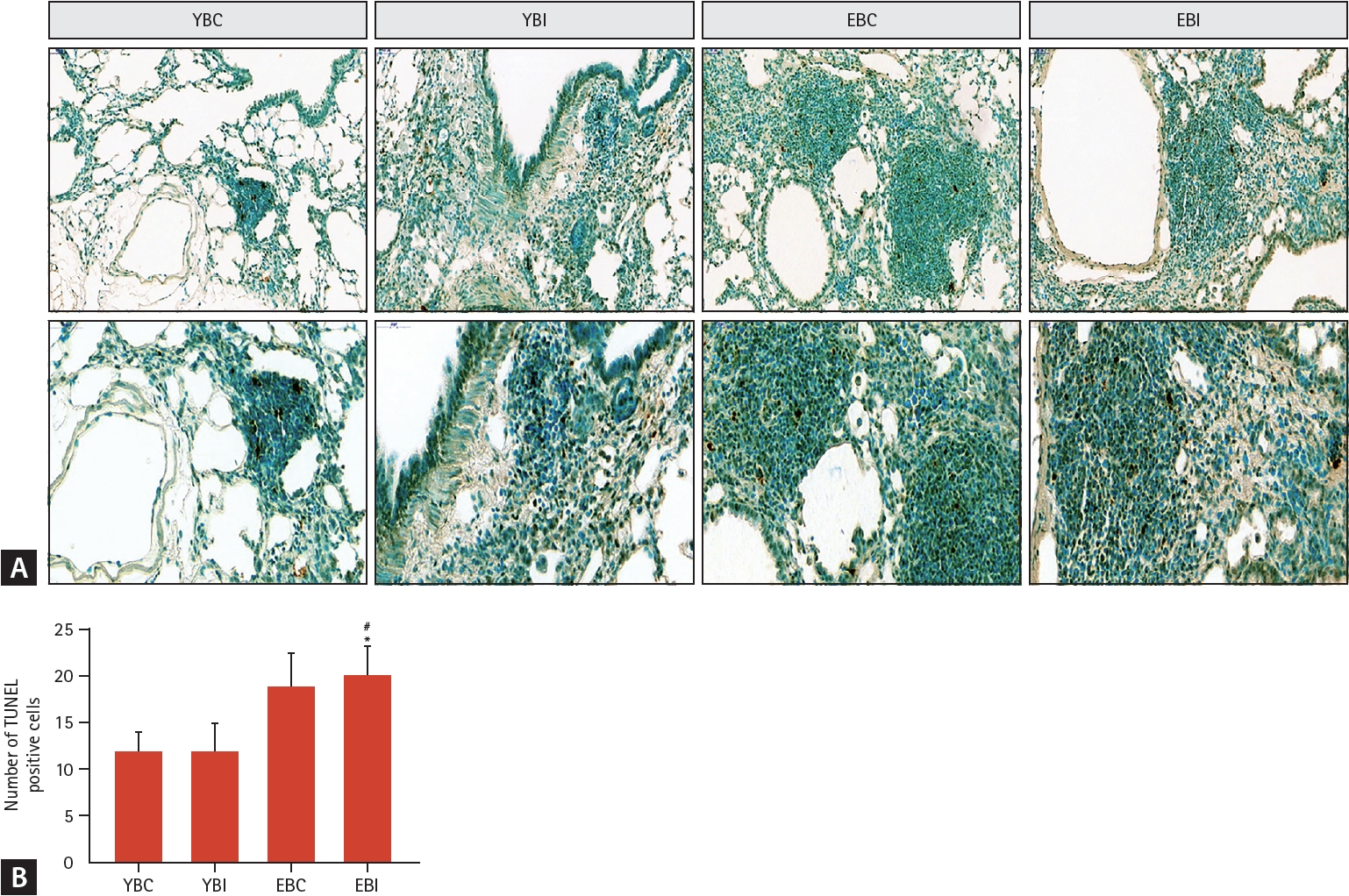
Effects of bleomycin and intermittent hypoxia on apoptosis in young and elderly mice. (A) Representative photomicrographs of lung tissues were stained with TUNEL assay kit to determine apoptotic cells (magnification, × 200). The images are enlarged in the lower panels (magnification, × 400). (B) The TUNEL-positive cells (dark brown-stained nuclei) were semi quantitatively assessed. Data represent the mean ± standard deviation. EBC, old + bleomycin + room air; EBI, old + bleomycin + intermittent hypoxia; YBC, young + bleomycin + room air; YBI, young + bleomycin + intermittent hypoxia. *p < 0.05 vs. YBC; #p < 0.05 vs. YBI.
DISCUSSION
Our study shows that IH augmented bleomycin-induced groups to exhibit severe inflammation, fibrosis, and oxidative stress. The elderly mouse IH bleomycin group showed more severe lung inflammation, fibrosis, and oxidative stress compared to the young mouse IH bleomycin group, suggesting that age is an important factor with regards to the effects of IH on IPF.
A higher prevalence of OSA in patients with IPF than in the general population has not yet been verified. Aging appears to be a common risk factor for both diseases. IPF is considered an age-related disease and OSA is frequently found in older adults, with its prevalence increasing two to three times in older adults compared to middle-aged adults [14]. Aging-related mechanisms, such as genomic instability, telomere attrition, epigenetic alterations, loss of proteostasis, deregulated nutrient sensing, cellular senescence, altered intercellular communications, and stem cell exhaustion have been suggested as potential hallmarks of lung fibrosis [15] and OSA [16]. Aging is associated with pharyngeal fat deposition, soft palate lengthening, muscle tone impairments, and pharyngeal sensory discrimination. This can result in older people having more severe upper airway obstruction [16]. Another common cause of IPF and OSA is reduced lung volume due to IPF. Reduced functional residual capacity increases respiratory effort owing to negative extrathoracic pressure and can lead to upper airway collapse [17]. Therefore, common risk factors for OSA and IPF, such as aging and reduced lung volume, need to be further investigated. OSA should be routinely screened in patients with IPF because it commonly coexists with IPF.
Recent results have suggested that OSA aggravates IPF and is closely associated with higher mortality and poor clinical outcomes [18]. However, the main aggravating factor could be hypoxia. In patients with IPF, chronic hypoxia is frequently observed as the disease progresses and can induce oxidative stress and systemic inflammation [19]. Patients with OSA often show repetitive episodes of apnea and hypopnea during the sleep cycle, which induces IH. Therefore, the co-occurrence of OSA and IPF can lead to IH and an increased duration of hypoxia, resulting in excessive systemic inflammation and overproduction of reactive oxygen species (ROS) [20]. Studies have shown that chronic IH aggravates bleomycin-induced lung injury in a mouse model by increasing neutrophilic inflammation, lung cell apoptosis, and collagen accumulation [21,22]. Our results are consistent with those of previous studies. Additionally, we report a novel finding stating that IH increased the severity of lung inflammation, oxidative stress, and fibrosis in the elderly mouse group compared to the younger group.
While this greater inflammatory response and severity have been recognized with age, the mechanism underlying enhanced inflammation is unclear. Potential contributors to enhanced inflammation in the bleomycin-induced elderly mice group could be changes in pulmonary function and physiology [15], underlying chronic systemic inflammation [23], or changes in the immune response [24]. In our study, we observed age-dependent increases in the levels of inflammatory cells and cytokines, lung fibrosis, and oxidative stress markers. It appears that IH enhanced the pro-inflammatory response, leading to lung fibrosis and oxidative stress, with a worse prognosis in the older mice group.
ROS has a very short lifespan, and ROS-related tissue destruction can be detected indirectly through the final products of lipid peroxidation, such as MDA [25]. The increased levels of MDA in the elderly groups demonstrate that the levels of oxidative stress are higher, especially in the EBI group compared to the younger groups. Common antioxidants include CAT, superoxide as CAT, and GSH-associated enzymes [26]. CAT mitigates oxidative stress by destroying cellular hydrogen peroxide to produce water and oxygen. CAT deficiency is associated with the pathogenesis of many age-related degenerative diseases; therefore, in our study, it was observed to be the lowest in the EBI group. The SOD and GSH levels did not differ among the groups in our study. We postulate that SOD activity is often reduced early; however, at later stages, it can increase to counteract elevated ROS levels. Therefore, this variation may have caused insignificant changes among the groups. Moreover, GSH levels can decrease in lung fibrosis and increase in normal lung tissue. These findings may have led to insignificant changes in the outcomes [26].
Furthermore, the characterization of immunosenescence markers, such as SA-β-gal, p21, and p53, during exposure to IH in both young and old mice showed that senescence markers were more evident in older mice with lung fibrosis exposed to IH. Among the two senescence pathways, our results are related to the p53-p21-pRb pathway [27]. Positive results related to the p16-pRb pathway were not observed in our results, which require further study. Our results showed that IPF is related to aging and cellular senescence, and that IH is associated with the augmentation of this process. Further studies are required to better understand the differential effects of aging.
Our study has several limitations. First, the IH mouse model, a widely used animal model for OSA, was not standardized, and the bleomycin dose and IH settings may have affected the outcomes. In addition, this model does not reflect other aspects of OSA such as sleep fragmentation, intrathoracic pressure swings, and intermittent hypercapnia. Second, further research on the mechanisms underlying the effects of aging on IH and IPF is required. Moreover, our results should be verified in human studies on older patients with IPF and OSA.
In conclusion, the bleomycin-induced IH-exposed older mice group showed significant increases in inflammation, fibrosis, and oxidative stress compared to the younger mice groups of the same characteristics. This suggests a potentially enhanced harmful impact of IH on elderly patients with IPF. Elderly patients with IPF and OSA may be more of a concern than younger patients with IPF. Further research on the subject matter is required.
KEY MESSAGES
1. IH, a key mechanism of OSA, augmented bleomycin-induced pulmonary fibrosis in a mouse model of severe inflammation, fibrosis, and oxidative stress.
2. The bleomycin-induced IH-exposed older mice group showed greater lung inflammation, fibrosis and oxidative stress than the other groups.
3. Chronological age may be an important factor to consider when studying the effects of OSA in patients with IPF.
Acknowledgements
This research was supported by the Research Foundation of Internal Medicine, The Catholic University of Korea in the program year of 2020, and by the Research Institute of Medical Science of The Catholic University of Korea, Eunpyeong St. Mary’s Hospital in 2020.
Notes
CRedit authorship contributions
Heayon Lee: formal analysis, visualization, writing - original draft, writing - review & editing; In Kyoung Kim: formal analysis, methodology; Jeonghyeon Im: data curation; Bae Suk Jin: writing - review & editing; Hwan Hee Kim: writing - review & editing; Sei Won Kim: conceptualization, visualization; Chang Dong Yeo: writing - review & editing; Sang Haak Lee: conceptualization, project administration
Conflicts of interest
The authors disclose no conflicts.
Funding
None